Attosecond-plasmonics with metal nanostructures
Over the past few years, plasmonics has been established as an important field of physics. This fact is largely due to its advantages in the controlling and manipulating of light on sub-wavelength scales.
A plasmon is a quantized density fluctuation of the electron gas in a solid. These oscillations can propagate inside the bulk or just along the surface, which is pointed out by referring to them as surface plasmons. If propagation is additionally restricted in the other spatial dimensions, we are dealing with localized particle plasmons. They can be induced in metallic nanoparticles, since there, the propagation is confined due to the small particle size.
In our ultrafast plasmonics laboratory at the University of Konstanz we generate and investigate surface plasmons as well as particle plasmons in micro- and nanostructures. Applying different techniques with a resolution on the nanometer scale, we can customize the structures for special applications. The equipment in our plasmonics laboratory enables us to optically excite plasmons in single nanostructures with intense ultrashort light pulses. The pulses can be tuned over a broad spectral range and are used to characterize the structures. It turns out that the possibility to produce nanostructures with high precision not only paves the way to manipulate light on the shortest spatial scales: also the temporal answer of plasmonic devices can be tailored. This is even feasible down to the attosecond regime, that is shorter than one single oscillation of the light field [2, 4].
Non-linear optics with metallic nanoantennas
One possibility to transmit electromagnetic waves in the radio frequency regime is by sending and receiving via antennas, whose dimensions are of the same order of magnitude as the wavelength. Scaling down the antenna concept to the visible and near-infrared spectral range (400 nm to 2 µm) was thus retarded until high–accuracy nanostructuring was properly developed over the last few years. Just as their counterparts for radiofrequencies, optical antennas are ideally suited for the conversion of freely propagating electromagnetic waves into localized energy and vice versa. They enable us to control light on sub-wavelength dimensions. Thus, the diffraction-limited possibilities of light manipulation by conventional free-space optics like lenses or mirrors are vastly expanded. Furthermore, the near-field amplification that can be achieved at optical antennas is also of great importance for advanced light-matter coupling. For instance, this goal can be achieved with hybrid systems consisting of semiconductor quantum dots coupled to antennas. Additionally, the highly nonlinear optical response of nanoantennas promises to hold a huge application potential.
Fabrication and characterization of metallic nanoantennas
Primarily, we use electron beam lithography for the fabrication of metallic structures with dimensions on the nanometer scale. This technique allows us to assemble a great variety of geometries in short time with a high precision of a few nanometers.
Figures 1a) to e) show SEM images of some structures. The following pictures present measurements of plasmonic modes that develop for either horizontal (f) to k)) or vertical polarization of the exciting light. One of our cooperation partners, the group of Ulrich Hohenester from the University of Graz, has computed the corresponding modes with the boundary element method, as shown in Figures 1i) to x).
Another method is based on polystyrene beads that form a colloidal mask on which gold is evaporated [1]. This approach enables us to produce small gold triangles that are mobile on the substrate. By means of an atomic force microscope, one can shift them and e.g. reduce their distance to investigate the coupling of their single modes. This coupling results in a redshift of the resonance frequency [1].

Optical setup for non-linear spectroscopy with single nanoantennas
For the optical excitation of plasmons in single metallic nanoantennas we use an erbium-doped femtosecond fiberlaser system (figure 2a)), generating ultrashort light pulses in combination with a pulse compression setup. At a repetition rate of 40 MHz, the near-infrared pulses can be tuned over more than one octave (0.9 to 2.1 µm central wavelength), which enables resonant excitation of antennas over a broad spectral range. Compression of the dispersive part of the spectrum yields pulses with a duration of 7.8 fs, just above the bandwidth limit. Using the entire spectrum, the generation of a single-cycle pulse can be demonstrated [3]. The laser pulses are coupled into an inverted microscope with almost no dispersion. Then they are focused onto a single nanoantenna (figure 2b)). The signal is spectrally resolved by a monochromator (MC) and detected by an avalanche photo diode (APD) or charge-coupled device (CCD) camera (figure 2c)).
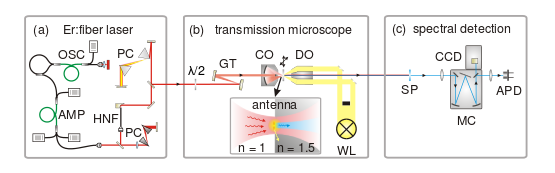

The setup offers perfect conditions for the investigation of the linear and non-linear response of a single nanoantenna with high spatial and temporal resolution. The field enhancement as well as the large third-order nonlinear susceptibility of Au support strongly non-linear effects, e.g. yielding an intense third-harmonic signal that can be seen with the naked eye. Tuning the exciting central wavelength trough the near-infrared spectral range shifts the color of the emitted light from blue to red.
In the time domain we study the dephasing of the collective electron oscillations. Therefore, we obtain the pulse durations via FROG (frequency resolved optical gating) [4] at the antenna (figure 3b and d) and on the surface of the bare substrate which responds instantaneously (Figure 3a and c). Combining the analysis of both measurements determines the dephasing time and its dependence on antenna volume and geometry [2, 4].

To optically excite delocalized plasmons, it is necessary that the plasmonic dispersion relation intersects with the photonic one. Only then the conversion of the k-vector and, therefore, the transformation is possible. This goal can be achieved at a sharp edge in a metal film. Figure 4a) shows a SEM (scanning electron microscope) image of a slit (width 100 nm) and a groove (width 200 nm) in gold [5]. Illuminating the groove with a laser generates plasmons that propagate towards the slit, where they are transformed back into light. This light passes the slit and can be detected beneath the bottom side. The distance the plasmons travel varies because of a small aperture angel between slit and groove. This causes an interference pattern of constructive and destructive superposition with light that is directly transmitted through the slit. The propagating plasmons respond very sensitively to changes in the metal film and thus are suited for sensitive sensors.
Our cooperation partner Vasily Temnov uses this arrangement for ultrafast pump-probe measurements, where a laser pulse influences the gold permittivity, thus changing the interference pattern distinctly [5]. In other experiments they use a magnetic field in multilayer structures of gold and cobalt to manipulate the plasmons [6].
Our group produces and characterizes the slit-groove-microinterferometer in cooperation with V. Temnov. For the fabrication we use focused ion beam etching, a technique that is available in the nanostructuring laboratory, where we achieve a precision of a few nanometers.
References
[1] J. Merlein, M. Kahl, A. Zuschlag, A. Sell, A. Halm, J. Boneberg, P. Leiderer, A. Leitenstorfer und R. Bratschitsch
"Nanomechanical control of an optical antenna"
Nature Photon. 2, 230 (2008)
[2] T. Hanke, J. Cesar, V. Knittel, U. Hohenester, A. Leitenstorfer und R. Bratschitsch
"Tailoring spatiotemporal light confinement in single plasmonic nanoantennas"
Nano Lett. 12, 992 (2012)
[3] G. Krauss, S. Lohss, T. Hanke, A. Sell, S. Eggert, R. Huber und A. Leitenstorfer
"Synthesis of a single cycle of light with compact erbium-doped fibre technology"
Nature Photon. 4, 33 (2010)
[4] T. Hanke, G. Krauss, D. Traeutlein, B. Wild, R. Bratschitsch und A. Leitenstorfer
"Efficient Nonlinear Light Emission of Single Gold Optical Antennas Driven by Few-Cycle Near-Infrared Pulses"
Phys. Rev. Lett. 103, 257404 (2009)
[5] V. V. Temnov, K. Nelson, G. Armelles, A. Cebollada, T. Thomay, A. Leitenstorfer und R. Bratschitsch
"Femtosecond surface plasmon interferometry"
Opt. Express 17, 8423 (2009)
[6] V. V. Temnov, G. Armelles, U. Woggon, D. Guzatov, A. Cebollada, A. Garcia-Martin, J.-M. Garcia-Martin, T. Thomay, A. Leitenstorfer und R. Bratschitsch
"Active magneto-plasmonics in hybrid metal–ferromagnet structures"
Nature Photon. 4, 107 (2010)